Silica nanoparticles: A review of their safety and current strategies to overcome biological barriers
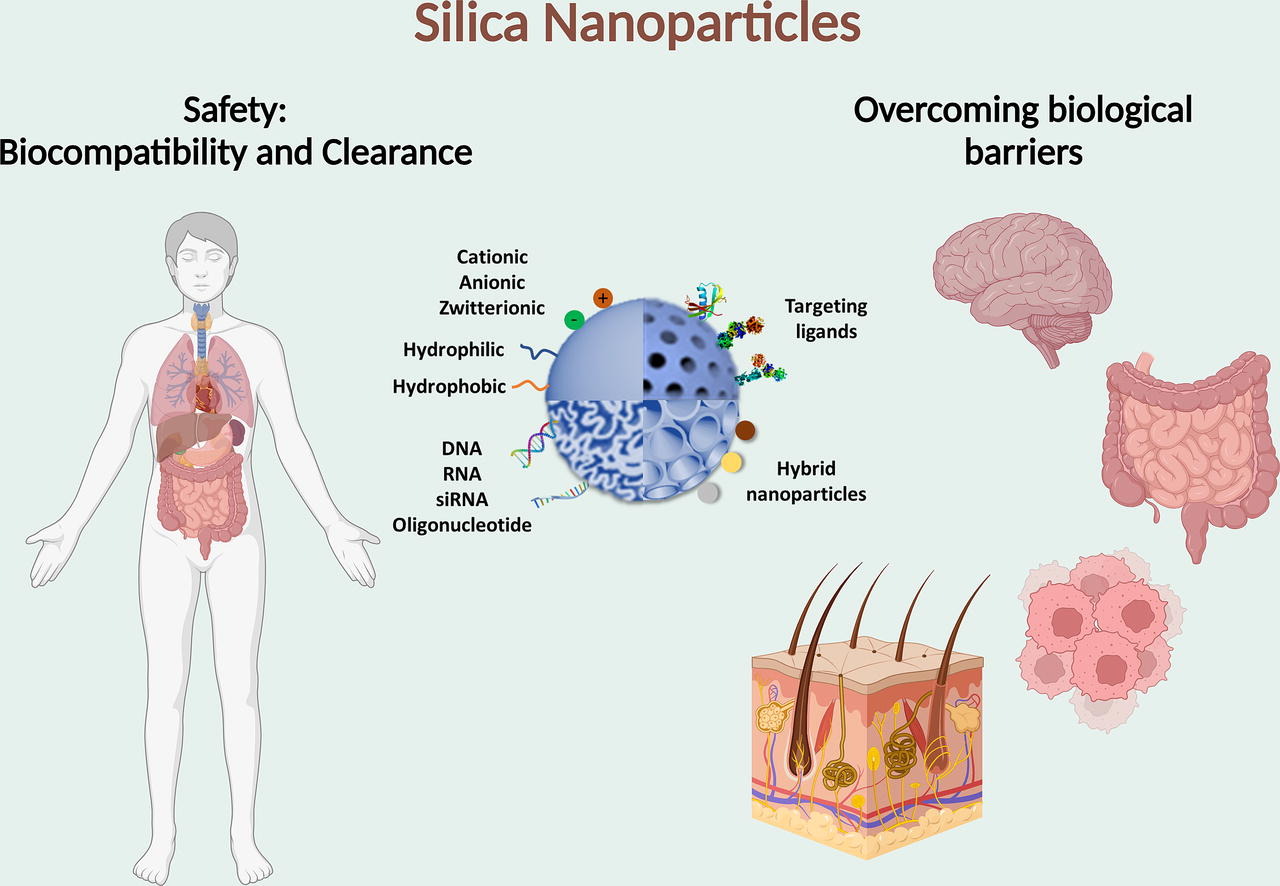
Abstract
Silica nanoparticles (SNP) have gained tremendous attention in the recent decades. They have been used in many different biomedical fields including diagnosis, biosensing and drug delivery. Medical uses of SNP for anti-cancer, anti-microbial and theranostic applications are especially prominent due to their exceptional performance to deliver many different small molecules and recently biologics (mRNA, siRNA, antigens, antibodies, proteins, and peptides) at targeted sites. The physical and chemical properties of SNP such as large specific surface area, tuneable particle size and porosity, excellent biodegradability and biocompatibility make them an ideal drug delivery and diagnostic platform. Based on the available data and the pre-clinical performance of SNP, recent interest has driven these innovative materials towards clinical application with many of the formulations already in Phase I and Phase II trials. Herein, the progress of SNP in biomedical field is reviewed, and their safety aspects are analysed. Importantly, we critically evaluate the key structural characteristics of SNP to overcome different biological barriers including the blood–brain barrier (BBB), skin, tumour barrier and mucosal barrier. Future directions, potential pathways, and target areas towards rapid clinical translation of SNP are also recommended.
Introduction
Nanotechnology-based diagnostic, sensing and drug delivery platforms have gained significant attention in the last few decades [1], [2]. When compared to conventional therapeutics, nanomaterials may offer many advantages, such as tailored drug delivery at the diseased site with reduced side effects and improved stability of formulations. The field of nanomedicine has progressed rapidly, and numerous nanoparticle-based delivery systems have been investigated preclinically and clinically [1], [3].
An optimal nanocarrier would possess a desired cargo loading capacity, show excellent biocompatibility and stability (on shelf and during transport), exhibit particle size and shape uniformity, and allow for targeted (organ and/or cell) cargo release. In light of this, there has been growing interest in developing porous inorganic materials which have unique properties such as high specific surface area, tuneable (surface) chemistry, and enhanced stability across biological barriers, when compared to organic nanoparticles such as polymers and liposomes [4]. Many inorganic or hybrid drug carriers have been investigated, such as silica, silicon, quantum dots, and metal–organic frameworks (MOFs). Among these, research on silica-based nanomaterials has witnessed not only an exponential growth in the number of publications but also a progressive transition from basic research towards clinical translation (Fig. 1) [5], [6], [7], [8], [9], [10], [11], [12], [13]. Silica-based nanoparticles, SNP, are very attractive due to their ease of synthesis, colloidal stability, tuneable particle size, ease of surface functionalization, biocompatibility, and potentially scalable synthesis [14], [15]. Importantly, SNP have been reported to enhance the stability of diverse cargo without affecting their specific chemical and physical functions [16], [17], [18], [19], [20].
One of the earliest SNP synthesis protocols, known as the Stöber process, was pioneered by Werner Stöber in 1968 (Fig. 1) [21]. Stöber particles are solid and monodisperse SNP with adjustable diameters in a wide range of 20–2000 nm. They are synthesized using sol–gel chemistry involving a silica precursor, such as tetraethylorthosilicate, in water and alcohol solutions containing ammonia [21]. In the last 50 years, many different types of SNP have been generated, having different particle sizes and shapes, as well as various pore structures [15], [22], [23], [24], [25], [26], [27]. Due to their versatile properties, SNP have now been used in a variety of applications such as food additives, excipients in pharmaceutical products and cosmetics, and as nutraceuticals [28]. For instance, the French cosmetic company L’Oréal uses SNP in many of their skincare products in order to improve skin texture and provide a matte finish [29]. Some sunscreens contain SNP, as they have a UV light scattering effect in addition to increasing dispersity of other UV blocking agents and thus improve the overall UV-attenuating efficacy of the formulations [30], [31], [32], [33].
Fig. 1: Schematic diagram representing the history and development of silica particles and silica nanoparticles (SNP) with different surface morphology and modifications.
Porous silica as a drug delivery agent is not new. In 1983, the amorphous colloidal and porous silicas synthesis was published, by Klaus Unger in Germany [34]. Unger et al., demonstrated that the porous silica can be used as a drug delivery and stabilizing agents. The early syntheses did not lead to the formation of nanoparticles, but rather non-uniform micron-sized particles. In the 1990′s, a new class of high surface area mesoporous silica material emerged, where surfactant assemblies served as supramolecular structure-directing agents, namely MCM-type ordered mesoporous silica. In 2001, Vallet-Regi et al., introduced MCM-41 as a potential drug carrier for the first time using ibuprofen as a model drug. In 2003,Lin et al was pioneered the synthesis particle tunable size and surface functionality, and achieved the control drug release property of SNP. [35]. Mesoporous materials of this class are attractive due to their porosity features which create high specific surface areas and pore volumes which are useful for loading of high payloads. Moreover, well-calibrated and ordered pores also allows good control of adsorption/release rates enabling best fit between drug size and pore sizes/ openings [36], [37]. Later developments along this direction allowed the synthesis of ordered mesoporous silicas with a narrow and adjustable pore size in the range 2–30 nm. [22], [38], [39] Importantly, the silica network is amorphous, which enhances the rate of biodegradation as compared to crystalline silica and silicates, which is also very important from a cytotoxicity point of view [40], [41], [42], [43]. Crystalline silica has a highly ordered and repetitive atomic structure, whereas silicon and oxygen atoms are arranged into periodic, hexagonal 6-membered rings via siloxane bonds that bridge between two silicon centres. This regular structure makes it more stable and less susceptible to chemical reactions or breakdown by biological processes. In contrast, amorphous silica lacks this long-range order, with a disordered and irregular structure, which makes it more prone to chemical and biological degradation[43], [44].
In 1999, a new hybrid class organic–inorganic silica-based material called periodic mesoporous organosilicas (PMOs) were developed independently by three research groups Ozin [45], Inagaki [46], and Stein [47]. Here, the solid wall of the porous material contained organic groups as part of the hybrid network, therefore the polarity of the pore walls differs from those of corresponding purely silica-based particles. Later, PMO-based nanoparticle systems, sometimes named as mesoporous organosilica nanoparticles (MON), were developed which have since become popular nanocarriers for drugs, genes and protein delivery as they may exhibit improved biodegradation and drug release profiles, as compared to the all-silica materials [48], [49], [50].
Medical uses of SNP for delivery of anti-cancer, diagnostic and theranostic purposes are now becoming prominent [5]. SNP such as MSN and MON offer the ability to encapsulate and precisely control drug release at specific site which can be triggered by changes to pH, reactive oxygen species, temperature, enzyme and light [20], [51], [52]. For example, tumour tissues are known to have an acidic pH environment, whereas normal tissues typically maintain a neutral pH. In response to this biological distinction, researchers have engineered a dual-responsive peptide, RGDFFFFC, designed to function as a ‘gatekeeper’ on the surface of MSN. Upon entry into the acidic tumours’ microenvironment, the drug-loaded nanoparticles benefit from enhanced uptake by tumour cells, facilitated by the exposure of the peptide targeting ligand. Subsequently, the presence of the intracellular redox signal, glutathione, within the tumour cells plays a pivotal role in triggering rapid drug release [53]. In addition, the adjustable porous structure of MSN and MON enables co-delivery of various therapeutic agents to the desired sites to combat the development of disease, such as cancer, and its subsequent resistance while also reducing the drug amount needed, thereby limiting off-target side effects [52], [54], [55], [56], [57]. SNP are also a suitable carrier system to deliver antimicrobial agents, as they can be modified for targeted delivery to site of infection in order to avoid toxicity associated with systemic circulation [20], [58], [59], [60], [61], [62]. In addition, unlike other nanocarriers such as gold, silver and polymeric nanoparticles, SNP such as MSN can have high drug loading capacity and improve the stability of active pharmaceutical ingredients by encapsulating the drug inside the pores, even including large antimicrobial peptides, which is important to both adequately treat the infection (especially biofilm) and prevent development of resistance [20], [62], [63], [64], [65], [66], [67], [68]. Nonetheless, a limitation in drug release arises from the interactions occurring between drug molecules and the silica surface, potentially resulting in incomplete drug release. The magnitude of this interaction is contingent upon the silica surface’s chemistry, which exhibits variations in response to pH levels. Moreover, it is influenced by the chemical composition and ionization states of the drug itself. This heightened adsorption, consequently, corresponds to a decreased extent of drug release [69]. Moreover, there has been high interest in using SNP for imaging and diagnostics, some formulations containing SNP have progressed to clinical trials in humans [5]. The traditionally-used gadolinium-based contrast agents used for magnetic resonance imaging (MRI) are highly toxic; the use of SNP as support for the Gd compounds can reduce the toxicity of these contrast agents by not only lowering the amount needed for high resolution MRI but by also limiting the leaching of Gd3+ ions into the circulation [70], [71], [72], [73]. Recently, the photostability of fluorescence imaging agents, such as indocyanine green (ICG) or cyanine dyes, has also been enhanced by loading into SNP due to the entrapment and stabilisation of the dye in nanopores [10], [18], [74]. Moreover, Chen et al. reported a PEGylated mesoporous silica nanoparticle loaded with J-aggregates of NIR fluorophore IR-140, allowed nanoparticles to absorb and emit shortwave infrared light [75]. This development enabled high resolution imaging in vivo with 980 nm excitation and 1000–1700 nm acquisition, and the formulation was stable for weeks in buffer[75]. Due to the controlled mesopores, diverse surface modification, mesopores silica nanoparticles have become a popular image agent supporting system including organic dyes, quantum dots and carbon dots, and their applications in bio-imaging have been extensively discussed elsewhere[76], [77], [78], [79], [80].
In addition to the advances related to the use of MSNs and MONs for drug delivery and bio-imaging, their use in overcoming biological barriers has recently been thoroughly investigated in various in vitro and in vivo models. Biological barriers such as skin, the blood–brain barrier (BBB), mucosal barriers in lungs and the intestinal tract are a part of the defense systems of the body that are involved in selective transport of substances. The nanoscale nature of SNP along with their specific properties such as size and shape, surface charge and functionality, stability and porosity generate complex physicochemical interactions with these biological barriers, in many instances leading to better transport of drugs across these hurdles. While numerous review articles cover SNP and their diverse applications in drug delivery [81], [82], [83], [84], [85] and theranostics [86], [87], an up-to-date review focusing on linking the material characteristics to the ability to overcome biological barriers with special emphasis on their rational design was needed.
Download the full article as PDF here Silica nanoparticles: A review of their safety and current strategies to overcome biological barriers
or read it here
Taskeen Iqbal Janjua, Yuxue Cao, Freddy Kleitz, Mika Linden, Chengzhong Yu, Amirali Popat, Silica nanoparticles: A review of their safety and current strategies to overcome biological barriers, Advanced Drug Delivery Reviews, 2023, 515115, ISSN 0169-409X, https://doi.org/10.1016/j.addr.2023.115115
See the webinar:
“Rational Selection of Cyclodextrins for the Solubilization of Poorly Soluble Oral Drugs”, 8. November 2023:
Get more information & register here for free:
