Continuous freeze-drying of messenger RNA lipid nanoparticles enables storage at higher temperatures
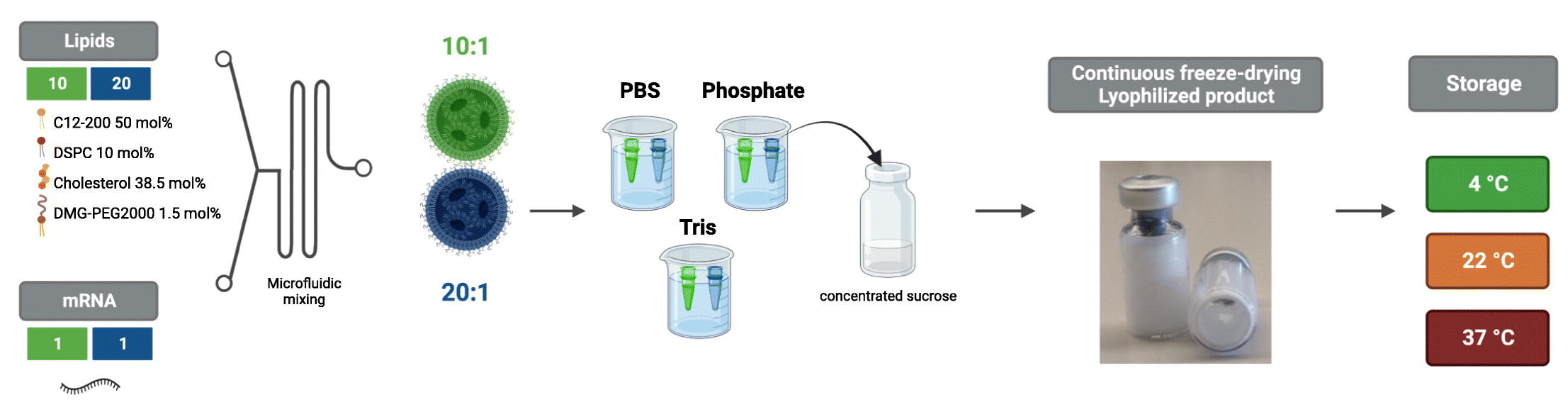
Messenger RNA (mRNA) lipid nanoparticles (LNPs) have emerged at the forefront during the COVID-19 vaccination campaign. Despite their tremendous success, mRNA vaccines currently require storage at deep freeze temperatures which complicates their storage and distribution, and ultimately leads to lower accessibility to low- and middle-income countries. To elaborate on this challenge, we investigated freeze-drying as a method to enable storage of mRNA LNPs at room- and even higher temperatures. More specifically, we explored a novel continuous freeze-drying technique based on spin-freezing, which has several advantages compared to classical batch freeze-drying including a much shorter drying time and improved process and product quality controlling. Here, we give insight into the variables that play a role during freeze-drying by evaluating the impact of the buffer and mRNA LNP formulation (ionizable lipid to mRNA weight ratio) on properties such as size, morphology and mRNA encapsulation.
We found that a sufficiently high ionizable lipid to mRNA weight ratio was necessary to prevent leakage of mRNA during freeze-drying and that phosphate and Tris, but not PBS, were appropriate buffers for lyophilization of mRNA LNPs. We also studied the stability of optimally lyophilized mRNA LNPs at 4 °C, 22 °C, and 37 °C and found that transfection properties of lyophilized mRNA LNPs were maintained during at least 12 weeks. To our knowledge, this is the first study that demonstrates that optimally lyophilized mRNA LNPs can be safely stored at higher temperatures for months without losing their transfection properties.
Introduction
During the coronavirus disease 2019 (COVID-19) pandemic, messenger RNA (mRNA) vaccines have emerged at the forefront. Less than one year after the pandemic outbreak, two mRNA vaccines were granted conditional marketing authorization for the prevention of COVID-19 as a first-of-its-kind vaccine technology, i.e. BNT162b2 (Comirnaty®) and mRNA-1273 (Spikevax®) developed by Pfizer/BioNTech and Moderna, respectively [1]. These vaccines both consist of N1-methylpseudouridine (m1ψ)-modified mRNA encoding the viral spike antigen, encapsulated in a lipid nanoparticle (LNP) which is composed of a proprietary ionizable cationic lipid, 1,2-distearoyl-sn-glycero-3-phosphocholine (DSPC), cholesterol, and a polyethylene glycol (PEGylated) lipid [2,3].
Despite their tremendous success in the COVID-19 pandemic, a drawback of mRNA vaccines is their poor stability, which consequently requires cold chain storage at low or even ultra-low temperatures [4,5]. To exemplify, Moderna’s Spikevax® vaccine and bivalent booster have a shelf-life of 9 months when kept at −50 °C to −15 °C and can only be stored for 30 days at refrigerator temperatures after thawing [6]. The formulation consists of mRNA LNPs suspended in Tris (20 mM) buffer containing 8.7 m/V% sucrose as cryoprotectant [7]. Comirnaty® even requires storage at −90 °C to −60 °C, preserving its stability for 12 months [8]. For its pediatric formulation and bivalent booster, Pfizer switched the buffer from phosphate-buffered saline (PBS) containing 10% sucrose to Tris (10 mM) 10% sucrose [9], which suggests that the buffer composition is important for the stability of mRNA LNPs. Indeed, due to this adaptation, the shelf-life at refrigerator temperatures could be increased from 1 month to 10 weeks while long-term storage at −60 °C to −90 °C is guaranteed for up to 12 months [10,11].
In comparison, other types of marketed COVID-19 vaccines can be stored at refrigerator temperatures, ranging from 6 months in the case of Vaxzevria® (an adenovirus vector vaccine by AstraZeneca/Oxford) [12], to 9 months for Nuvaxovid® (a protein-based vaccine by Novavax) [13], up to even 11 months for Jcovden® (an adenovirus vector vaccine by Janssen) [14]. Notwithstanding the ability of these vaccines to be stored at higher temperatures than mRNA vaccines, they still require cold chain logistics which demand well-trained personnel to maximally prevent the wastage of inadequately stored vaccines [15]. However, when vaccines need deep freeze storage, such as the current mRNA vaccines, cold chain logistics become even more challenging as this demands specialized infrastructure including expensive ultracold freezers [16]. For these reasons, vaccines requiring (deep freeze) cold chain logistics not only demand extensive organization but also become less accessible for low- and middle-income countries [15].
A potential solution to improve the shelf-life of mRNA LNP vaccines at higher temperatures is to develop a lyophilized formulation. Lyophilization or freeze-drying is a method to eliminate water from a heat-labile formulation. In a dehydrated state, hydrolysis is less likely to take place while also molecular mobility is drastically reduced, slowing down degradation reactions [17]. Lyophilization comprises a freezing phase and a drying phase, both of which are known to induce stresses to lipid-based nanoparticle formulations [[18], [19], [20]]. Therefore, lyoprotectants are typically added to protect liposomal and RNA LNP formulations during freeze-drying [19,20]. Two theories have been proposed to explain the working mechanism of lyoprotectants, namely the water replacement theory and the vitrification theory. The water replacement theory states that sugars can interact with phospholipids (in case of e.g. liposomes) via hydrogen bonds, so as to replace water molecules during freeze-drying and conserve the structure of the lipid membrane [18]. On the other hand, the vitrification theory proposes that sugars can freeze-concentrate and subsequently undergo vitrification to form a glass matrix with high viscosity, preventing changes in lipid membranes [18]. Recently, a few reports were published that investigated the role of lyoprotectants during freeze-drying of RNA LNPs [[21], [22], [23]]; some other studies showed that mRNA LNPs could be successfully lyophilized and stored without really digging into a mechanistic understanding [24,25]. Although these prior studies have demonstrated the feasibility of lyophilization of RNA LNPs, a thorough investigation of the factors that could play a role in freeze-drying of RNA LNPs has not yet been discussed. For instance, as freezing rate, ionic strength, and changes in pH are frequently reported to impact the outcome of lyophilization, they should also be considered when optimizing a lyophilization process for mRNA LNPs [19].
In previous studies on this topic, batch freeze-drying of RNA LNPs was performed [26]. It should be noted, however, that such batch processes have some drawbacks, including a long (and expensive) process and drying time, mostly ranging from 1 to 7 days [27]. Furthermore, vial-to-vial variability is inherent to the process and can lead to altered critical quality attributes (CQAs) of the individual vials [28]. Also, it is not possible to perform any in-process controls of individual vials [28]. Finally, scaling batch freeze-drying processes from lab-scale to pilot-scale to production-scale is very time-consuming as well. Because of these limitations and the general incentive toward continuous manufacturing by regulatory authorities [29], a continuous process for the freeze-drying of RNA LNPs was evaluated in this study. More specifically, we explored spin-freezing and radiative drying, as developed by Corver et al. [30] In this method, vials are spun along their longitudinal axis during the freezing step, causing the fluid to spread over the lateral surface of the glass vial. As a consequence, a frozen product is obtained with a larger surface area allowing a faster heat- and mass transfer and thus shortened drying time [28]. Subsequently, during the drying phase, the vials move along a series of infrared (IR)-heaters in a low-pressure environment. In contrast to batch freeze drying, this technology results in a drastic reduction of drying time, improved process control, and 100% product quality control [28].
Here, we present a method to lyophilize mRNA LNPs by continuous freeze-drying based on spin-freezing. The nucleoside-modified mRNA LNPs comprised the ionizable lipidoid C12–200 but resembled the COVID-19 mRNA vaccines with respect to helper lipids and lipid molar ratios. First, we evaluated the effect of buffer type (PBS, phosphate, and Tris at different buffer capacities) and lipid:mRNA weight (wt) ratio on the lyophilization of mRNA LNP formulations. To this end, we compared lyophilized mRNA LNPs to freshly prepared control mRNA LNPs in terms of size, polydispersity (PdI), zeta potential, encapsulation efficiency, and transfection efficiency. We demonstrated that a sufficiently high lipid:mRNA wt ratio and an optimal buffer (phosphate or Tris) were necessary to prevent leakage of encapsulated mRNA, and thus to maintain the functionality of the mRNA LNPs. We then studied the stability of optimally lyophilized mRNA LNPs stored at various temperatures (4 °C, 22 °C, and 37 °C) for a period of 12 weeks. When stored at higher temperatures, compared to non-lyophilized mRNA LNPs, the stability of lyophilized mRNA LNPs was drastically improved. Finally, we validated the functionality of lyophilized mRNA LNPs in vivo, by measuring luciferase expression of fresh and lyophilized firefly luciferase (fLuc) encoding mRNA LNPs. We believe that this study provides important insights into lyophilization of mRNA LNPs and reveals potential for the storage of mRNA LNPs at higher temperatures, which may ease the logistics and transportation of these novel vaccines.
Read more
Sofie Meulewaeter, Gust Nuytten, Miffy H.Y. Cheng, Stefaan C. De Smedt, Pieter R. Cullis, Thomas De Beer, Ine Lentacker, Rein Verbeke, Continuous freeze-drying of messenger RNA lipid nanoparticles enables storage at higher temperatures, Journal of Controlled Release, Volume 357, 2023, Pages 149-160, ISSN 0168-3659, https://doi.org/10.1016/j.jconrel.2023.03.039.