Extreme Extensibility in Physically Cross-Linked Nanocomposite Hydrogels Leveraging Dynamic Polymer–Nanoparticle Interactions
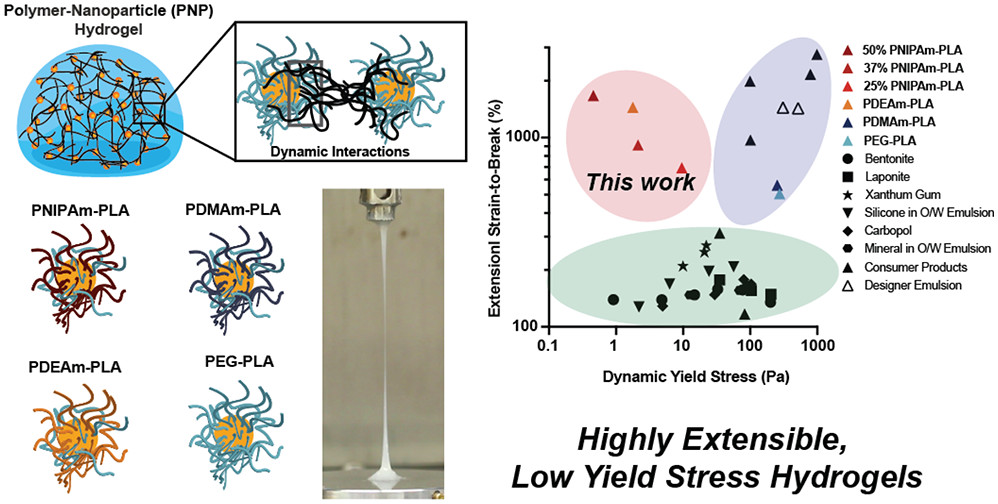
Abstract
Designing yield stress fluids to exhibit desired functional properties is an integral challenge in many applications such as 3D printing, drilling, food formulation, fiber spinning, adhesives, and injectable biomaterials. Extensibility in particular has been found to be a highly beneficial characteristic for materials in these applications; however, few highly extensible, high water content materials have been reported to date. Herein we engineer a class of high water content nanocomposite hydrogel materials leveraging multivalent, noncovalent, polymer–nanoparticle (PNP) interactions between modified cellulose polymers and biodegradable nanoparticles. We show that modulation of the chemical composition of the PNP hydrogels controls the dynamic cross-linking interactions within the polymer network and directly impacts yielding and viscoelastic responses. These materials can be engineered to stretch up to 2000% strain and occupy an unprecedented property regime for extensible yield stress fluids. Moreover, a dimensional analysis of the relationships between extensibility and the relaxation and recovery time scales of these nanocomposite hydrogels uncovers generalizable design criteria that will be critical for future development of extensible materials.
Introduction
Results and Discussion
Fabrication of Polymer–Nanoparticle Hydrogels
In this study, we aimed to design a series of nanoparticles with different polymer coronas to investigate how modulation of the resulting PNP interactions impacts the mechanical properties of the resulting PNP hydrogels. In particular, we sought to assess whether changing the interaction dynamics could yield diverse mechanical properties and enhance extensibility of these materials. To explore this design space, we synthesized a series of diblock copolymers: (i) poly(N-isopropylacrylamide)-b-poly(lactic acid) (PNIPAm–PLA), (ii) poly(diethylacrylamide)-b-poly(lactic acid) (PDEAm–PLA), (iii) poly(dimethylacrylamide)-b-poly(lactic acid) (PDMAm–PLA), and (iv) PEG–PLA (Figure 1b). We chose to focus on polyacrylamide-based polymers on account of the broad array of nonionic and water-soluble monomers that are commercially available and exhibit tunable degrees of hydrophobicity. (29) We selected the four diblock copolymers used in this study for several key physicochemical differences. First, PNIPAm and PDEAm are relatively hydrophobic polymers with log P ∼ 1, evidenced by their observable lower critical solution behavior at modest temperatures, while PDMAm and PEG are more hydrophilic with log P ∼ 0 (log P values estimated from PubChem). Chi parameters, if available, would also demonstrate differences in hydrophobicity for these polymers. Second, while all four polymers are capable of accepting hydrogen bonds, only PNIPAm is capable of hydrogen bond donation.
Polyacrylamide-based copolymers with relatively low molecular weight PLA blocks (<5 kDa) have been synthesized previously with tin catalysts that are often unsuitable for biological applications due to toxicity concerns and challenging removal of the catalyst postsynthesis. (30-33) Here we aimed to create diblock copolymers with relatively high molecular weight PLA blocks (20 kDa) to support preparation of highly stable nanoparticles using more biocompatible synthetic methods. To synthesize polyacrylamide-based copolymers, we leveraged copper-free click chemistry and fractional precipitation techniques (Figures 2a, S1, and S2). (34-36) Polyacrylamide derivatives were first synthesized by using reversible addition–fragmentation chain transfer (RAFT) polymerization techniques (37) affording controlled molecular weights and low dispersity. A bicyclononyne derivative (BCN-amine) was then conjugated to the end-group of the polymers. PLA was polymerized from azidoethanol by using an organocatalytic ring-opening polymerization technique with 1,8-diazabicycloundec-7-ene (DBU) as a catalyst. (38) These two polymers were then coupled together to form diblock copolymers by using copper-free strain-promoted azide–alkyne cycloaddition (SPAAC). (35,39) Fractional precipitation in solvents of varying polarity were then performed to isolate the desired product from residual homopolymer to yield monodisperse diblock copolymers (**D < 1.12) with a water-soluble block of 5–6 kDa and a PLA block of 17–20 kDa (Figure 2b, Table S1, and Figure S3).
Polymer–Nanoparticle Hydrogel Shear Rheological Properties
In addition to oscillatory testing, flow testing was also performed to assess static and dynamic apparent yield stress behavior in these materials. The static yield stress is defined as the stress at which the material begins to flow upon an increase in stress, while the dynamic yield stress is the minimum stress required for maintaining flow. (43,44) PNP hydrogels comprising hydrophobic PNIPAm- and PDEAm-based NPs exhibited lower static yield stress behavior than gels comprising hydrophilic PDMAm- and PEG-based NPs. Similarly, the dynamic yield stress values observed for materials comprising hydrophobic NPs were much lower than those of materials comprising hydrophilic NPs, but all PNP hydrogels demonstrated Herschel–Bulkley behavior characteristic of yield stress fluids with clear plateaus at low shear rates. (3,45) In all materials, the static yield stress was much higher than the dynamic yield stress, suggesting these materials are thixotropic and have a time scale associated with microstructural reformation. (43,44) Because of these time-dependent effects, both measurements should be considered apparent yield stress values. Critically, this apparent yield stress behavior is only observed in PNP hydrogels, as the HPMC-C12 solutions alone do not exhibit prominent yield stress behavior (Figure S7). These findings suggest that the hydrophobicity of the NP corona greatly affects the polymer–nanoparticle interactions with the HPMC-C12, providing an avenue for facile modulation of the macrostructural properties and performance of PNP hydrogels.
Examining Extensibility
Tunability of Polymer–Nanoparticle Interactions
Probing Recovery Time Scales of PNP Hydrogels
To examine the time scale associated with recovery, or thixotropy, we performed stress-overshoot experiments on all formulations at shear rates in the flow regime. (59) Stress overshoot behavior, while not fully understood, has been found to be characteristic of yield stress fluid behavior. (60,61) The stress overshoot during shear was measured after various wait times while compensating for a measured recoverable strain after yielding between each step (Figure 6a and Figure S13). (59) McKinley and co-workers reported that the growth of this overshoot is representative of a characteristic recovery or restructuring time scale (Figure 6c–e). (62) We find that an analogous time scale (τS) can be found by fitting the stress overshoot growth data according to

where σ is the stress overshoot, t is the wait time, and A is a scaling constant.
Examining the Origins of Extreme Extensibility

The PNP hydrogels comprising hydrophobic PNIPAm- and PDEAm-based NPs allow us to uniquely access improved extensibility in these materials, while still maintaining their yield stress behavior. These hydrophobic polymers likely form a more compact (i.e., less extended and hydrated) corona on the NPs than the hydrophilic polymers do, thereby reducing the interaction between the NPs and the HPMC-C12. These trends indicate that modulation of the relaxation behavior (i.e., De according to relaxation time scales) can be used to design yield stress fluids to meet application-specific material properties. (15) These findings are highly relevant to fields such as 3D bioprinting or fiber spinning, where extrusion fidelity is highly related to the extensible characteristics of the material. (2)
Download the full study as PDF here Extreme Extensibility in Physically Cross-Linked Nanocomposite Hydrogels Leveraging Dynamic Polymer–Nanoparticle Interactions
or read it here
Extreme Extensibility in Physically Cross-Linked Nanocomposite Hydrogels Leveraging Dynamic Polymer–Nanoparticle Interactions Abigail K. Grosskopf, Joseph L. Mann, Julie Baillet, Hector Lopez Hernandez, Anton A. A. Autzen, Anthony C. Yu, and Eric A. Appel Macromolecules Article ASAP
DOI: 10.1021/acs.macromol.2c00649