Novel Therapeutic Hybrid Systems Using Hydrogels and Nanotechnology: A Focus on Nanoemulgels for the Treatment of Skin Diseases
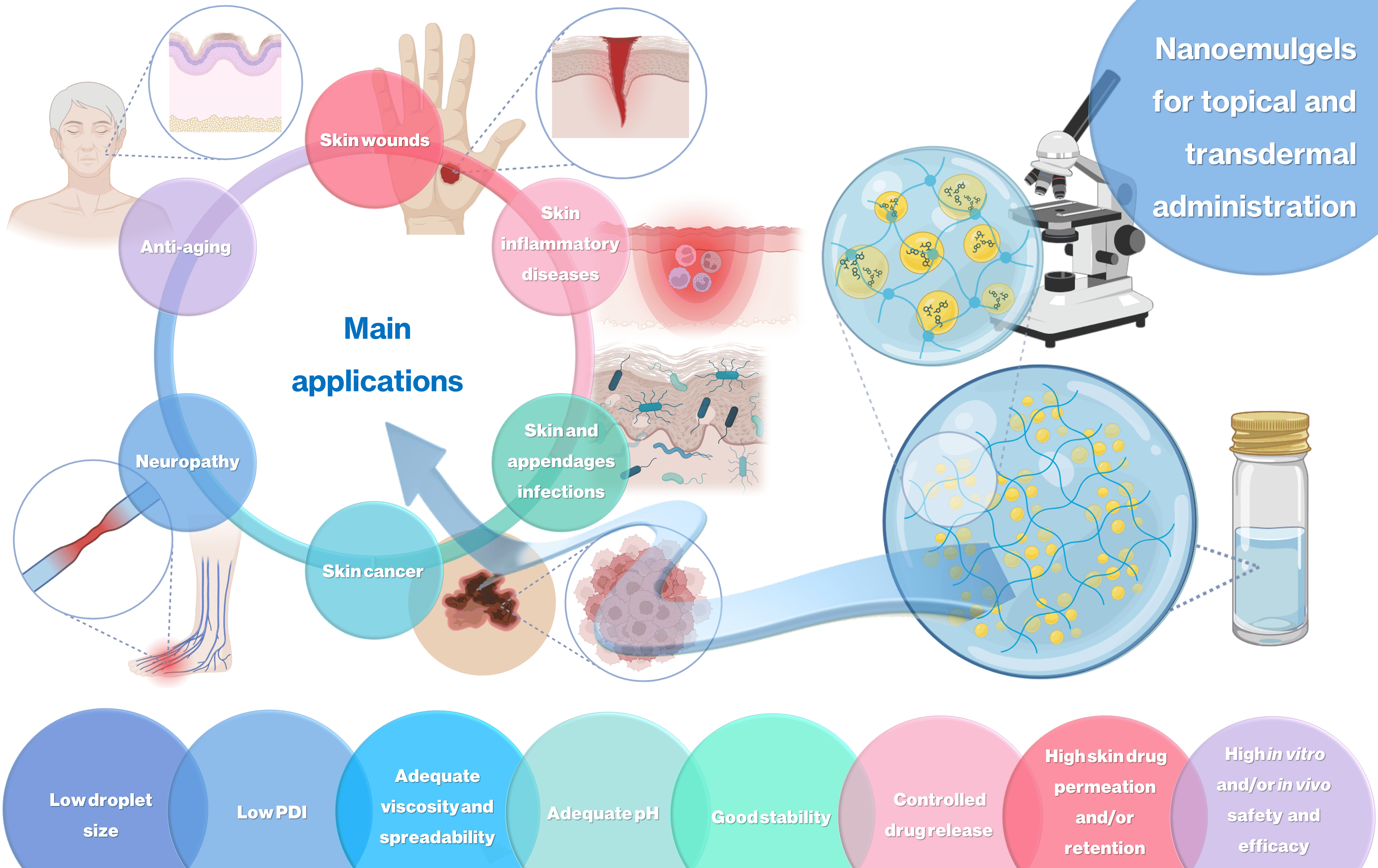
Abstract
Topical and transdermal drug delivery are advantageous administration routes, especially when treating diseases and conditions with a skin etiology. Nevertheless, conventional dosage forms often lead to low therapeutic efficacy, safety issues, and patient noncompliance. To tackle these issues, novel topical and transdermal platforms involving nanotechnology have been developed.
This review focuses on the latest advances regarding the development of nanoemulgels for skin application, encapsulating a wide variety of molecules, including already marketed drugs (miconazole, ketoconazole, fusidic acid, imiquimod, meloxicam), repurposed marketed drugs (atorvastatin, omeprazole, leflunomide), natural-derived compounds (eucalyptol, naringenin, thymoquinone, curcumin, chrysin, brucine, capsaicin), and other synthetic molecules (ebselen, tocotrienols, retinyl palmitate), for wound healing, skin and skin appendage infections, skin inflammatory diseases, skin cancer, neuropathy, or anti-aging purposes.
Developed formulations revealed adequate droplet size, PDI, viscosity, spreadability, pH, stability, drug release, and drug permeation and/or retention capacity, having more advantageous characteristics than current marketed formulations. In vitro and/or in vivo studies established the safety and efficacy of the developed formulations, confirming their therapeutic potential, and making them promising platforms for the replacement of current therapies, or as possible adjuvant treatments, which might someday effectively reach the market to help fight highly incident skin or systemic diseases and conditions.
Download the full article as PDF here: Novel Therapeutic Hybrid Systems Using Hydrogels and Nanotechnology
1. Introduction
1.1. The Skin: Properties and Advantages and Limitations as a Drug Delivery Route
The skin consists of three primary layers: the epidermis, the dermis, and the hypodermis [1,2,3]. The epidermis, the outermost layer, is divided into several sublayers, with the deepest layer being known as the stratum basale (also called the basal layer). This layer contains rapidly dividing basal cells that continually undergo mitosis to replace the cells lost from the skin’s surface. As these basal cells divide and mature, they gradually move upwards toward the skin’s surface [4,5]. Above the stratum basale lies the stratum spinosum, which provides strength and support to the epidermis. The cells in this layer have spiny projections that interlock with neighboring cells, enhancing tissue integrity [6,7]. Further up is the stratum granulosum, where the cells begin to produce large amounts of keratin and other proteins. As these cells mature, they form flattened, granular layers, preparing to become the outermost protective barrier of the skin [7,8]. Finally, we have the stratum corneum, the outermost layer of the epidermis. This layer is composed of tough, flattened, and fully keratinized cells known as corneocytes. These corneocytes are continuously shed and replaced by new cells from the lower layers. The stratum corneum acts as a formidable barrier, preventing the entry of pathogens and chemicals, and excessive water loss [9,10,11]. Beneath the epidermis lies the dermis, a thicker and more complex layer of the skin, primarily composed of connective tissue, which includes collagen and elastic fibers, providing structural support and elasticity to the skin. The dermis houses blood vessels, nerves, hair follicles, sebaceous glands, and sweat glands. It also contains sensory receptors and specialized cells like Merkel cells, responsible for detecting touch and pressure [12,13,14]. Lastly, the hypodermis, or subcutaneous tissue, forms the deepest layer of the skin. It is mainly composed of adipose tissue (fat) that acts as an insulator and cushion, regulating body temperature and providing protection to underlying organs and structures [15,16].
Several factors can affect the properties of the skin. Environmental factors such as ultraviolet (UV) radiation from the sun can cause skin damage, premature aging, and increase the risk of skin cancer. Pollution, chemicals, and harsh weather conditions can also impact the skin’s health and appearance [17,18,19]. Additionally, lifestyle choices, such as diet, smoking, and alcohol consumption, can also influence the skin’s elasticity and overall health. Hormonal changes, stress, and certain medical conditions may also lead to skin issues, which can consequently evolve into pathological conditions, such as acne, eczema, and psoriasis, among others [20,21,22,23]. Understanding the intricate organization of the skin’s layers and their functions is essential for maintaining healthy skin. Proper care, protection from harmful environmental factors, and a balanced lifestyle can contribute to the overall health and well-being of this remarkable organ [24,25].
Furthermore, the fact that the skin, as the body’s largest external organ, serves as a protective barrier against various external factors, makes drug delivery on or through the skin a significant challenge, since the skin exhibits a very low or even nonexistent permeability to most drug molecules [26,27,28]. Factors such as stratum corneum composition, hydration, anatomical site, and individual variations contribute to the overall high complexity of skin drug permeation, and the understanding of the skin’s characteristics is essential for optimum drug delivery, in both topical and transdermal administration [24,29,30].
Transdermal and topical drug administration are both methods of delivering medications to the body through the skin. However, there are some key differences between the two [31,32]. Transdermal application refers to the delivery of medications through the skin, and into the bloodstream, allowing active ingredients to have systemic effects since they are meant to be distributed throughout the body. Hence, the formulation is designed to penetrate the skin’s surface and reach the bloodstream [33,34]. It is commonly used for medications that require slow, continuous release into the bloodstream over an extended period of time, and often used for systemic conditions such as hormone replacement therapy, pain management, or nicotine replacement therapy, among other applications [35,36,37]. Drugs are usually delivered through patches, gels, or creams, specifically designed to facilitate absorption through the skin and controlled release into the bloodstream [38,39,40,41]. On the other hand, topical administration involves applying medications directly to the skin’s surface to exert local effects on the area of application. In this case, the drug is meant to stay primarily at the site of application and will probably not be designed to penetrate deeply into the skin’s layers and reach the bloodstream [42,43,44]. Hence, this administration route is typically used for localized conditions, such as skin infections, rashes, inflammation, and other skin-related issues [45,46,47]. Formulations are designed to target specific areas without affecting the entire body, and come in various forms, including creams, ointments, lotions, sprays, and foams, depending on the intended application [48,49,50].
Nevertheless, although topical and transdermal drug delivery have gained significant interest due to their numerous advantages, including noninvasiveness, easy administration, and possibility of a localized therapy, conventional pharmaceutical forms often lack an answer to the many challenges that drug delivery on or through the skin presents, such as drug permeation and/or retention, drug metabolism by the skin’s enzymes, or effective solubilization of hydrophobic drugs [33,51,52,53]. Here, novel approaches using nanotechnology for drug encapsulation and delivery can be the answer.
1.2. Nanotechnology for Efficient Skin Drug Delivery: A Special Focus on Nanoemulsions and Nanoemulgels
Nanosystems are generally characterized as being structures in the nanosize range which are capable of encapsulating drug molecules for improved drug delivery. Several different types of nanosystems have been developed over the years, each with their own specific composition and characteristics (Figure 1) [54,55,56,57].
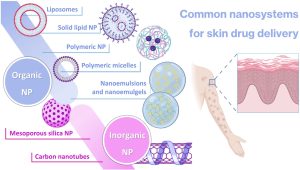
Nanoparticles (NPs) are the most common general type of nanosystems, with the term typically referring to particles within the size range of 1 to 100 nm [58,59]. Their adjustable physicochemical properties, such as size, shape, and surface characteristics, contribute to tailored and improved physiological performance, when compared to conventional pharmaceutical forms, generally leading to more effective treatment and minimization of the side effects [60,61,62,63]. NPs also hold significant potential for targeted delivery of active ingredients to specific locations, in contrast to conventional formulations which usually require the administration of larger quantities of active ingredients in order to reach a therapeutically effective response, and often fail to target the desired area of interest, resulting in an extensive and potentially harmful penetration of the drugs into healthy tissues, hence causing systemic side effects [60,64,65]. Key considerations include these particles’ size and structure, which is essential to optimize their performance and minimize potential harm, with surface modification by different types of substances, such as polymers and other molecules, being important in what concerns stability, specificity, and therapeutic effectiveness [66,67,68]. NPs can be divided into various subcategories, including organic and inorganic nanocarriers [69,70,71].
Inorganic NPs include mesoporous silica nanoparticles (MSNs), which are nanoscale structures composed of silica, with large surface areas and well-defined pores. Their ordered porous structure, with uniform pore sizes ranging from 2 to 50 nm, allows for efficient encapsulation and controlled release of active substances [72,73]. The large surface area of MSNs provides a space for active ingredient loading, leading to high drug-loading capacity and enhanced therapeutic efficacy, but despite these advantages, MSN synthesis requires precise control over particle size, pore site, and surface chemistry, which can be complex and time-consuming [74,75,76]. Carbon nanotubes (CNTs) are another type of inorganic nanocarrier, being cylindrical structures composed of carbon atoms arranged in hexagonal lattice, producing a large surface area that offers significant loading capacity for efficient encapsulation of therapeutic agents [77,78]. They possess unique advantages, such as high mechanical strength, exceptional electrical conductivity, thermal stability, protection of incorporated substances from degradation, controlled release capacity, and targeting ability [79,80,81]. These properties, combined with their nanoscale dimensions, make CNTs attractive candidates for therapeutic applications. Nevertheless, they have quite significant disadvantages, especially their poor water solubility and potential for toxicity [82,83,84]. Given the potential toxicity of inorganic nanoparticles, among other disadvantages, organic nanocarriers have been preferred for various applications [85,86].
There are many types of organic nanocarriers, all having in common high biocompatibility with the human body, an essential criterion for drug delivery in order to minimize toxicity and side effects [87,88]. Liposomes, the first to be developed, and also the first to effectively reach the pharmaceutical market, are spherical nanocarriers with single (small or large unilamellar vesicles) or multiple (multilamellar vesicles) bilayered membranes, formed of natural or synthetic lipids, which enclose an aqueous core [89,90,91,92]. They have showed a number of advantages compared to conventional systems, including enhanced drug delivery, drug protection from degradation, improved bioavailability, and even prolonged half-life in the blood circulation when functionalized with specific polymers (such as polyethylene glycol (PEG)) on their surface (stealth or PEGylated liposomes) [82,93,94]. On the other hand, solid lipid nanoparticles (SLNs) are formed by dispersing melted solid lipids in water, while emulsifiers are employed to stabilize the dispersion. These nanocarriers provide a lipophilic lipid matrix that facilitates the dispersion or dissolution of lipophilic drugs, providing controlled drug delivery, biocompatibility, high drug payload, and improved bioavailability of poorly water-soluble drugs [82,95,96]. Nevertheless, some important disadvantages of SLNs reside in their inability to encapsulate hydrophilic drug molecules, and stability issues have been reported, including burst release of the drugs during storage [97,98]. Another relevant nanocarrier subcategory is the polymeric micelles (PMs), self-assembled nanostructures formed by amphiphilic block copolymers in aqueous solutions. These nanosystems consist of a hydrophobic core, inside which hydrophobic drugs can be encapsulated, and a hydrophilic shell, stabilizing the micelle, which is made possible by the unique structure of block copolymers. These structures can also be surface-functionalized with targeting ligands to achieve specific delivery to target cells or tissues [99,100,101]. On the other hand, also composed of polymers, polymeric nanoparticles (PNPs) are nanocarriers that are fabricated using biocompatible polymers, typically poly (lactic-co-glycolic acid) (PLGA), PEG, chitosan, or polycaprolactone (PCL), amongst others. PNPs have the ability to encapsulate and protect various types of drugs including small molecules, proteins, peptides, and nucleic acids. The encapsulation also improves their stability, and controls their release kinetics, which allows for sustained or targeted drug delivery [102,103,104]. However, both PMs and PNPs have been reported to exhibit toxicity, slow clearance, formulation instability issues due to aggregation, and low drug-loading capacity [102,105,106].
Additionally, all mentioned nanocarrier types have complex preparation methods, which are time-consuming, costly, and often lead to scale-up issues, while also many times not being environment-friendly due to the use of organic solvents and the need for high energy amounts during production [107,108,109].
Given the overall stability, toxicity, low drug-loading, and problematic preparation issues that are seen with other nanocarrier types, nanoemulsions and nanoemulgels have arisen as advantageous solutions with high potential. Nanoemulsions are kinetically stable biphasic dispersions of two immiscible phases, an oil phase and a water phase, which are combined with surfactants and/or cosurfactants to increase their stability. Two main types of nanoemulsions exist, water-in-oil (W/O) or oil-in-water (O/W), with the latter being the most common [110,111,112]. Usually, O/W nanoemulsions contain from 5% to 20% oils/lipids, with this amount increasing up to 70% when the nanoemulsion is W/O. The type of the oil used in the formulation of nanoemulsions depends on the active substances that are intended to be solubilized, with the selection often being performed based on a solubility criterion (oils that solubilize the active substance the best) [111,113]. On the other hand, surfactants are amphiphilic molecules, which are used to stabilize the nanoemulsions, to reduce the interfacial tension and prevent the aggregation of the internal phase droplets. They usually have an ability to absorb quickly at the oil–water interface, and provide steric, electrostatic, or dual electro-steric stabilization.
Cosurfactants may be used as surfactant complements to strengthen the interfacial film, if they fit suitably in areas which are structurally weaker [114,115,116]. There is an ongoing effort in both industrial preparations and scientific works to ensure that the components which are used in the development of nanoemulsions be strictly nontoxic, generally-regarded-as-safe (GRAS) excipients, in order to ensure maximum biocompatibility and decrease the propensity for side effects potentiated by the formulation [117,118]. Nanoemulsions can be produced by high-energy, low-energy, or low- and high-energy combination methods [117,119]. High-energy emulsification methods depend on mechanical devices, which use energy for creating powerful disruptive forces to reduce the size of the formed droplets. Ultrasonicators, microfluidizers, and high-pressure homogenizers can be used, including on an industrial level. These methods have the advantage of being able to nanoemulsify almost any oil, but the dependence on instrumental techniques is associated with high costs and the generation of high temperatures, which might not be feasible for all formulation components (for example, thermolabile drugs) [120,121].
Hence, low-energy methods, such as phase inversion or spontaneous emulsification, are the most advantageous, since it is the energy stored in the system that is used to produce the ultrafine droplets, leading to lower production costs and an easy application [122,123]. One of the most used low-energy methods is the phase inversion temperature method, to take advantage of changes in the aqueous/oil solubility of surfactants in response to temperature fluctuation. This will include the conversion of a W/O to an O/W nanoemulsion, or the reverse, via an intermediary bicontinuous phase. The change of temperature from low to high causes the opening and reversal of interfacial structure, which leads to phase inversion. However, this method has a substantial disadvantage, which is that it cannot be used for thermosensitive drugs [124,125,126]. Hence, the most beneficial low-energy method ends up being spontaneous emulsification, in which the components are usually just mixed with each another, resorting to manual mixing or low-energy mixers (such as mechanical stirring or vortex stirring).
This, of course, only happens when the right components are mixed in the right proportions, with the oils, surfactants and cosurfactants having to be miscible with one another, and with reasonably high amounts of surfactants/cosurfactants being valuable for maximum formulation stabilization, homogeneity, and low droplet size [127,128,129]. Regardless of the used preparation method, nanoemulsions’ droplet size usually varies between 20 and 500 nm, hence being small and responsible for these formulations’ clear or hazy appearance [117,130]. This type of nanosystem can be used in many different dosage forms, such as creams, sprays, gels, aerosols, or foams, and various administration routes, such as intravenous, oral, intranasal, pulmonary, ocular, topical, or transdermal drug delivery [111,131,132]. They have several relevant advantages, such as a high solubilization capacity, great long-term physical stability, which reduces the propensity for conventional destabilization mechanisms to occur (such as creaming, coalescence, or Ostwald ripening), and a very large surface area available for drug absorption to occur [133,134,135]. Nevertheless, for certain applications, nanoemulsions might not be the ideal dosage form, since they tend to be fluid and do not usually have bioadhesion capability, which can lead to a short retention time of the preparation at the site of administration, an important parameter in transdermal and topical administration. In order to tackle these issues, nanoemulsions can be transformed into nanoemulgels [136,137].
Nanoemulgels are hybrid colloidal systems composed of nanosized oil droplets dispersed in an aqueous gel matrix, combining the properties of both nanoemulsions and hydrogels, which solve important issues such as spreadability and skin retention of the preparations by increasing their viscosity [136,138]. The methodology for preparing a nanoemulgel entails the creation of a gel-based formulation using common and already described emulsification techniques, similar to those that are used in the preparation of nanoemulsions [139,140]. As topical or transdermal administration systems, they function as drug reservoirs, facilitating the controlled release of the drug from the inner phase to the outer phase, and subsequently onto the skin. Aside from controlled drug delivery, nanoemulgels exhibit several advantages over alternative topical formulations, which include compatibility with the skin, high viscosity, adhesiveness, good spreadability, and long-lasting therapeutic effects [139,140]. In what concerns common excipients that are used as part of a nanoemulgel’s composition, oil selection is usually dependent on the intended hydrophobicity, viscosity, permeability, and stability in the formulated nanoemulsion, but oils from natural sources, such as oleic acid, are commonly used, or chemically modified oils with medium chain mono-, di-, or tri-glycerides, such as Capryol® 90, Miglyol® 812, or Labrafac™ [141,142,143]. Additionally, there are several categories of surfactants, and among the most used are cationic surfactants (such as amines and quaternary ammonium compounds, or lecithin), anionic surfactants (such as sodium bis-2-ethylhexylsulfosuccinate, or sodium dodecyl sulfate), zwitterionic surfactants (such as phospholipids), and nonionic surfactants (such as Tweens, Lauroglycol® 90, Cremophor® EL, or Cremophor® RH 40) [137,144,145]. Frequently used cosurfactants include propylene glycol, PEG 400, ethyl alcohol, or Transcutol®, with alcohol-based cosurfactants hence being the most preferred due to their capability to split between oil and water phases, thereby improving their miscibility [139,146,147]. Lastly, there are different types of gelling agents used in nanoemulgel formation, including natural gelling agents such as bio-polysaccharides (such as pectin, carrageenan, alginic acid, locust bean gum, or gelatine), derivates of bio-polysaccharides (such as xanthan gum, starch, dextran, or acacia gum), as well as semisynthetic (such as hydroxypropyl cellulose, ethyl cellulose, and sodium alginate) and synthetic polymers (such as carbomers or poloxamers) [136,137,148].
When developing a novel nanoemulgel, several properties have to be evaluated, in order to assess whether the preparation has optimum characteristics for the intended application, including droplet size, polydispersity index (PDI), zeta potential, pH, rheological properties, stability, bioadhesion, spreadability, in vitro drug release, ex vivo drug permeation, toxicity potential, and in vitro and/or in vivo assessment of the therapeutical potential of the developed formulation for the intended purpose. This review focuses on the latest advances regarding the development of novel nanoemulgels for transdermal or topical administration, for the treatment of several highly impactful diseases and conditions (Figure 2), such as skin wound healing, skin and appendage infections, skin inflammatory diseases, skin cancer, neuropathy, and skin aging. A critical analysis is performed regarding formulation composition and preparation, and all relevant characterization parameters, in order to assess the true potential of these formulations as novel functional platforms for drug delivery onto or through the skin (summary in Table 1).
Table 1. Summary of the most relevant analyzed information of each included study, namely, disease intended to treat, encapsulated molecule(s), main formulation composition, droplet size, PDI, ZP, pH, main in vitro and/or in vivo therapeutic efficacy-related results, and corresponding reference.
Disease Intended to Treat | Encapsulated Molecule(s) | Main Formulation Composition | Droplet Size (nm) | PDI | ZP (mV) | pH | Main In Vitro and/or In Vivo Therapeutic Efficacy Related Results | Reference |
---|---|---|---|---|---|---|---|---|
Skin cancer | Chrysin | Capryol® 90, Tween® 80, Transcutol® HP, Pluronic® F127, water | <300 | 0.26 | −15 | NR | Strong antiproliferative effect in human and murine melanoma and human epidermoid carcinoma cell lines | [142] |
Skin cancer and psoriasis | Leflunomide | Capryol® 90, Cremophor® EL, Transcutol® HP, Pluronic® F-127, water | 98.7 to 280.92 | 0.2 to 0.3 | −7.8 | NR | Significant antimelanoma activity by inducing apoptosis and inhibiting tumor cell proliferation in melanoma cells; potent antipsoriatic activity by inhibiting human keratinocyte proliferation and reducing proinflammatory cytokine levels | [146] |
Skin wound healing | Atorvastatin | Liquid paraffin, Tween® 80, propylene glycol, carboxymethyl cellulose, water | 100 to 200 | <0.300 | −20 to −30 | 7.6 to 7.8 | Positive effect on wound healing, showing reduced inflammation, increased angiogenesis, and marked improvement in the skin’s histological architecture, after topical application on rat skin for 21 days | [149] |
Skin wound healing | Eucalyptus oil | Black seed oil, Tween® 80, Span® 60, propylene glycol, Carbopol® 940, water | 139 ± 5.8 | <0.450 | −28.05 | 5 to 6 | Significant improvement in wound healing in a rabbit model, with almost complete wound contraction after a 15-day period | [150] |
Skin wound healing | Tocotrienols and naringenin | Capryol® 90, Solutol® HS15, Transcutol® P, Carbopol® 934 or Carbopol® 940, water | 145.6 ± 12.5 | 0.452 ± 0.03 | −21.1 ± 3.32 | 4.9 to 5.3 | NR | [151] |
Skin wound healing | Thymoquinone | Black seed oil, Kolliphor® EL, Transcutol® HP, Carbopol® 940, water | 40.02 to 99.66 | 0.052 to 0.542 | −26.7 to −30.6 | 5.53 ± 0.04 | Accelerated wound closure in an in vivo rat wound model, evidenced by reduced wound size, enhanced re-epithelization, and increased collagen deposition | [152] |
Skin wound healing | Curcumin | Labrafac™ PG, Tween® 80, PEG 400, Carbopol® 940, water | 49.61 to 84.23 | 0.10 to 0.23 | −15.96 ± 0.55 to −20.26 ± 0.65 | 5.53 ± 0.03 | Significant wound-healing activity in Wistar rats, with almost complete wound healing after 20 days, with reduced inflammatory cells and extensive collagen fiber production | [153] |
Skin infections | Miconazole nitrate | Olive oil, almond oil, Tween® 80, Span® 80, Carbopol® 940, water | 170 | 0.193 | <−30 | NR | Significant antifungal activity against selected fungal strains (Candida albicans) | [154] |
Skin infections | Omeprazole | Olive oil, Span® 80, Tween® 80, chitosan, water | 369.7 ± 8.77 | 0.316 | −15.3 ± 6.7 | 6.21 ± 0.21 | Substantial antibacterial effects against both Gram-negative bacteria (Escherichia coli, Klebsiella pneumoniae, Pseudomonas aeruginosa) and Gram-positive bacteria (Staphylococcus aureus) | [155] |
Skin infections | Ebselen | Captex® 300 EP/NF, Kolliphor® ELP, dimethylacetamide, Soluplus®, Aquaphor, water | 54.82 ± 1.26 | NR | −1.69 | NR | Potent antifungal activity against multi-drug-resistant Candida albicans and Candida tropicalis | [156] |
Skin appendage infections (nails) | Ketoconazole | Labrafac™ Lipophile WL1349, Polysorbate 80, PEG 400, Carbopol® Ultrez 21, glycerin, methylparaben, thioglycolic acid, aminomethyl propanol, water | 77.52 ± 0.92 | 0.128 ± 0.035 | −5.44 ± 0.67 | 6.4 ± 0.24 | Significant antifungal activity against clinical isolates of dermatophytes, namely, Trichophyton rubrum and Candida albicans | [157] |
Skin infections | Fusidic acid | Myrrh oil, Tween® 80, Transcutol® P, CMC, water | 116 to 226 | NR | NR | 6.61 ± 0.23 | Substantial antibacterial activity, with significant inhibition zones against Staphylococcus Aureus, Bacillus subtilis, Enterococcus faecalis, Candida albicans, Shigella, and Escherichia coli | [158] |
Skin cancer | Imiquimod and curcumin | Oleic acid, Tween® 20, Transcutol® HP, Carbopol® 934, water | 78.39 | 0.254 | −18.7 | 5.5 | Did not lead to the appearance of psoriasis-like symptoms after topical application to mice | [159] |
Skin inflammatory diseases | Meloxicam | Eucalyptus oil, Tween® 80, PEG 400, Transcutol® P, distilled water | 139 ± 2.31 to 257 ± 3.61 | NR | NR | 6.58 ± 0.21 | Confirmed anti-inflammatory effects with reduced inflammation percentage in in vivo study | [160] |
Skin inflammatory diseases | Brucine | Myrrh oil, Tween® 80, PEG 400, ethyl alcohol, carboxymethyl cellulose, water | 151 ± 12 | 0.243 | NR | 6.2 ± 0.2 | Anti-inflammatory effects (significant decrease in inflammation) and antinociceptive effects (reduction in writhing movements) in an animal model, after topical application | [161] |
Diabetic neuropathy | Capsaicin | Eucalyptus oil, Tween® 80, propylene glycol, ethanol, isopropyl alcohol, Carbopol® 940, water | 28.15 ± 0.24 | 0.27 ± 0.05 | NR | NR | Significant in vivo efficacy in alleviating mechanical allodynia | [162] |
Skin aging | Retinyl palmitate | Capryol® 90, Captex® 355, Kolliphor® EL, Transcutol® HP, Carbopol® 940, glycerin, water | 16.71 | 0.015 | −20.6 | 5.53 ± 0.06 | NR | [163] |
Download the full article as PDF here: Novel Therapeutic Hybrid Systems Using Hydrogels and Nanotechnology
or read it here
Sghier, K.; Mur, M.; Veiga, F.; Paiva-Santos, A.C.; Pires, P.C. Novel Therapeutic Hybrid Systems Using Hydrogels and Nanotechnology: A Focus on Nanoemulgels for the Treatment of Skin Diseases. Gels 2024, 10, 45. https://doi.org/10.3390/gels10010045